Navigating Targeted Protein Degraders: Advanced Analytical Tools
eBook
Published: October 6, 2023
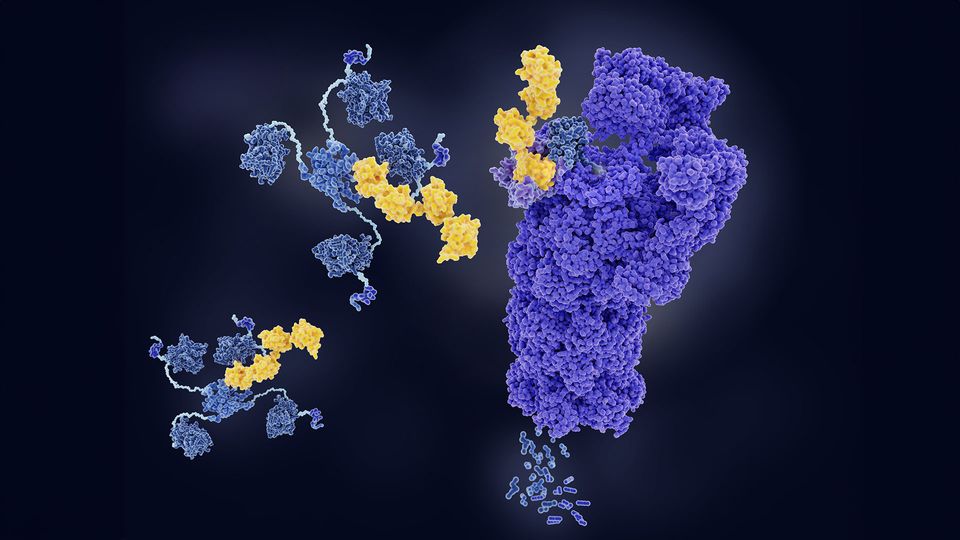
Credit: iStock
Over 85% of the human proteome lacks clear binding sites that can be targeted by standard pharmaceuticals. This renders many protein targets “undruggable”.
Targeted protein degradation (TPD) tackles this issue by directly regulating cellular machinery, enabling the destruction of specific, otherwise undruggable proteins. However, conventional TPD therapeutic discovery pipelines can be time-consuming, complex, and miss key off-target effects.
This eBook explores how recent advances in trapped ion mobility spectrometry (TIMS) have revolutionized TPD for a faster, more accurate development processes.
Download this eBook to discover:
- How proteomic approaches can help create more effective TPD therapeutics
- The advantages of TIMS over conventional mass spectrometry
- How to reduce drug attrition with early identification of off-target effects
NAVIGATING TARGETED
PROTEIN DEGRADERS
Advanced Analytical Tools
for High-Throughput Proteomics
Innovation with Integrity
Foreword
Targeted protein degradation (TPD) involves the selective degradation of disease-causing proteins using small molecule compounds and has shown promise in overcoming the limitations of traditional small molecule inhibitors and antibody-based therapies.
Recent advancements in mass spectrometry technologies have enabled more sensitive and accurate analysis of complex protein samples, and one such advancement is trapped ion mobility spectrometry (TIMS). TIMS is a high-resolution separation technique that can improve the performance of mass spectrometry instruments by separating ions based on their size, shape and charge.
When applied to TPD development, TIMS offers a powerful tool for characterizing the activity of a degrader drug on a protein of interest and the surrounding proteins in the cell. By quantifying proteins and related modifications, researchers can confirm the drug candidate’s mechanism of action and make early predictions about possible side effects.
In this eBook, we will explore the potential of TIMS applied to the discovery of novel TPD drugs and development. We will delve into the principles and applications of these techniques and explore the latest advancements in mass spectrometry technology that make TIMS an invaluable tool in proteomic analysis. Ultimately, our goal is to provide a comprehensive guide for researchers and scientists interested in leveraging TIMS to develop more effective TPD therapeutics.
Table of Contents
Exploring Targeted Protein Degradation 4
Infographic - Targeted Protein Degradation: The Future of Drug Development 10
NEOsphere Biotechnologies: Breaking the Barriers of TPD Drug Discovery 11
Plexium: Developing Next-Generation TPD Drugs 15
TPD as a Powerful Research Tool in Basic Biology and Drug Target Discovery 18
Development of NanoLuc-Targeting Protein Degraders and a
Universal Reporter System to Benchmark Tag-Targeted Degradation Platforms 20
Introduction
The field of drug discovery has grown rapidly
in the last few decades, with a worldwide
market worth over $70 billion.1 Despite this,
there are on average only 50 new drugs
approved each year.2 Thus, a multitude of
diseases are still lacking effective therapeutics.
Drug discovery and development
face many challenges, including low rates
of translation from animal models, heterogeneity
of patient populations and unknown
pathophysiology.3 Yet, one of the major
challenges is tackling the undruggable proteome.
Over 85% of the human proteome
is considered “undruggable”, meaning that
these proteins lack clear binding pockets
or enzymatic sites that can be targeted by
standard pharmacological agents.4,5 Many
of these undruggable proteins play key roles
in human disease, making them of great
therapeutic interest and driving the search
for innovative technologies. One such
innovation is targeted protein degradation
(TPD). In TPD, small molecule ligands are
used to directly regulate the cell’s protein
homeostasis and target specific proteins for
degradation.6 Since the concept was first
proposed in 1999, TPD-based technologies
have become a powerful tool to explore
cellular mechanisms and target formerly
undruggable proteins.7
Targeted protein degradation
In order to maintain protein homeostasis – or
proteostasis – in the cell, both the production
and degradation of proteins must be
regulated. Protein degradation can occur in
response to specific internal and external
signals or to remove faulty or damaged
proteins from the cellular environment.8 In
mammalian cells, there are two pathways
that degrade proteins to their constituent
amino acids: the Ubiquitin-proteasome
system (UPS) and the autophagy lysosome
Exploring Targeted Protein
Degradation
In TPD, small molecule ligands are used to
directly regulate the cell’s protein homeostasis
and target specific proteins for degradation
Targeted Protein Degraders 4
What is TPD?
E3 ligase POI
Molecular glue Ubiquitin
Available for
another round
of poly-Ubq of
the POI
Poly-ubiquitin
Proteasomal
degradation
A
Proteasomal
degradation
E3 ligase
E3 ligase
recruiting
arm
Linker POI
recruiting
arm
PROTAC
POI
Ubiquitin Poly-ubiquitin
Available for
another round
of poly-Ubq of
the POI
B
pathway (ALP). In the UPS, cytosolic and
nuclear proteins are marked for degradation
by the repeated attachment of the small
polypeptide, ubiquitin. The resulting multiubiquitin
chain targets the protein for degradation
by a large protease complex known
as the proteasome.8,9
Susceptibility to UPS-mediated degradation
can be increased by particular protein elements
called degrons (e.g., short amino acid
sequences, structural motifs, exposed amino
acids). Degrons can be introduced to proteins
of interest (POIs) to deliberately induce their
controlled downregulation – also known as
targeted protein degradation.10 Conditional
degrons, i.e., those activated or inhibited by
stimuli such as temperature or the expression
of another protein, enable a far more rapid,
specific and reversible method of protein
knockdown than genetic techniques such as
CRISPR-Cas9 or RNA interference.11
The development of small molecule
degrader systems has opened up degrons
to therapeutic applications, whereby specific
disease-associated proteins can be removed
by the cell’s own machinery. Several types
of these degrader systems have been
established, including proteolysis targeting
chimeras (PROTACs), lysosome-targeting
chimeras (LYTACs), antibody-based
PROTACs (AbTACs) and molecular glues.
Of these, PROTACs and molecular glues
have seen the most success so far.7 Both
systems rely on the recruitment of an E3
ligase to the POI, forming a ternary complex
(Figure 1). The E3 ligase then catalyzes the
transfer of ubiquitin (Ub) molecules from the
Ub-conjugating enzyme (E2) to the POI. This
action is repeated, leading to the polyubiquitination
of the POI and its subsequent
targeting to the proteasome for destruction.
7,12 Although they both exploit the same
process, there are clear differences between
PROTACs and molecular glues. PROTACs
are heterobifunctional molecules, consisting
of an E3 recruiting ligand and a POI targeting
warhead, connected by a flexible linker.
The PROTAC makes two distinct small
molecule-protein interactions to bring the
POI and E3 ligase together.13 In comparison,
molecular glues are a single molecule that
facilitate ubiquitination by turning the POI
into neo-substrate for the E3 ligase, thereby
enhancing protein–protein interactions
between the two.14
In 2019, ARV-110 became the first PROTAC
to enter clinical trial and subsequently gave
the first clinical proof of concept for PROTACs
against cancer targets.14,15 Since then,
several more protein degraders have entered
clinical testing, with 18 different molecules
in either phase I or phase II trials.16 However,
the full scope of potential TPD therapies is
yet to be discovered. For example, from a
family of 600 E3 ubiquitin ligases, less than
10 have so far been exploited for TPD.17,18
Figure 1
A) The mode of action of molecular glues for TPD. B) The mode of action of PROTACs.
In 2019, ARV-110 became the first PROTAC to
enter clinical trial and subsequently gave the
first clinical proof of concept for PROTACs
against cancer targets
Targeted Protein Degraders 5
What is TPD?
Optimizing TPD discovery and design
Until recently, the discovery of many novel
degraders has either been serendipitous (such
as the discovery that thalidomide functions
as a molecular glue), or through screenings
of extensive small-molecule libraries.19 This
is particularly inefficient for PROTACs as the
structural complexities of the ternary complex
make linkers difficult to design.20 PROTACs
also display properties that lie outside the
well-established standards of drug properties
(e.g., molecular weight), resulting in further
development challenges and limited routes
of administration.21 Rational design methods
implementing deep learning models and simulations
are currently being used to discover
new PROTACs by predicting function and
ligand binding properties.20 However, screening
and development methods must still be
improved if TPD is to become a key player in
the sphere of drug discovery.
Current approaches to characterization and
validation of protein degraders focus on a
stepwise assessment of multiple criteria
including cell permeability, target and E3
ligase engagement, polyubiquination and
target degradation.22 Assessment includes
phenotypic assays for target engagement
(e.g., cellular thermal shift assay or CETSA),
end-point assays (e.g., fluorescence-based
protocols) and mass spectrometry. Following
a phenotypic screen, techniques such as
CETSA are used to determine target engagement
and deconvolution. CETSA detects
changes in the thermal stability of a protein
induced by ligand binding, which confirms
the engagement of the degrader with the
POI and ligase.23 Traditionally, the detection
method in CETSA was a western blot, which
limited throughput and demanded high-quality
antibodies – which are not always available
for the POI.23 CETSA is now routinely coupled
to mass spectrometry (MS). This enables
opportunities for higher-throughput methods
of detection, though still requires extensive
optimization and provides little detail beyond
target engagement.24,25 Analysis of the formation
and structure of the ternary complex
provides valuable information for degrader validation.
This can be assessed using end-point
methods such as time-resolved fluorescence
energy transfer (TR-FRET), but these methods
again result in little data beyond target
quantification.26 Real-time, live-cell methods
– such as the recently developed Nanoluc
bioluminescence resonance energy transfer
(NanoBRET) assay – enable kinetic measurements
of target interactions throughout the
proteasomal pathway to a greater degree of
accuracy.12,26,27 However, even these methods
fail to account for whole-cell, off-target
effects of degraders – a major stumbling
block in the TPD development pathway.28
TIMS tunnel 1 TIMS tunnel 2
Isolation Fragmentation
(~3 ms / precursor)
Parallel
accumulation
PASEF scan
Figure 2
Trapped ion mobility spectrometry and the PASEF method.
Targeted Protein Degraders 6
What is TPD?
Assessment of off-target effects is crucial in
any drug development workflow. Failure to
address this early in the process can result
in high levels of drug attrition at later stages.
Off-target effects in TPD are often reflected
as changes in protein levels, which is poorly
assessed by the standard in vitro binding
assays and transcriptomics analyses used
in classic drug development.29,30 Hence, a
different approach is essential to ensure
safety for targeted protein degraders. Mass
spectrometry (MS) has long been used in
the screening and optimization of protein
degraders, as it offers sensitive detection
without requiring specific antibodies or tags.
MS is also the preferred detection method
for CETSA assays but can be difficult to
precisely deconvolute on- and off-target
effects.31 Recently, however, the use of
quantitative MS has enabled a proteomic-
based approach to TPD development.
Global proteomics analysis allows for the
examination of POI abundance and validation
of degrader selectivity. Furthermore, it
enables the simultaneous assessment of
any off-target or cell-wide effects, and therefore
a more complete approach to degrader
characterization.26
Expanding the capabilities of proteomics
approaches
MS-based proteomics approaches are
rapidly gaining traction in the TPD field for
analysis of on- and off-target effects. These
methods can significantly increase the
efficiency of optimizing TPD therapeutics,
and have already been successfully used in
several studies to this effect.30,32,33 However,
the comprehensive detection and quantification
of proteomes by MS alone still presents
multiple challenges, due to the complexity
of samples and the breadth of potential peptides.
34 Many MS techniques are also still
limited by time-consuming sample preparation,
detection sensitivity and, most importantly,
throughput.34,35,36 In response, recent
developments in this area have focused on
increasing fragmentation speed, without
losing sensitivity.34
Trapped ion mobility spectrometry (TIMS)
is a gas phase ion separation technique that
has been shown to tackle these challenges
in TPD development. In TIMS, ions are
propelled into a tunnel by a gas flow. The
drag force of the gas flow is opposed by an
electrical field, which traps ions in place at
certain points in the tunnel according to their
mobility (Figure 2). Changing the intensity
of the electrical field allows the selective
release of the ions for analysis based on
m/z and Collisional Cross Section (CCS) of
the molecules.34,36,37 Coupling TIMS with
a quadrupole time of flight (Q-TOF) mass
spectrometer increases sensitivity, speed
and characterization confidence in proteomics
workflows.34,35,38 TIMS also enables the
parallel accumulation–serial fragmentation
(PASEF®) method. In PASEF, ion packets
are accumulated in the front of the TIMS,
then separated by both their m/z and CCS
using trapped ion mobility. Signal-to-noise
is improved from the added dimension of
gas-phase separation, enabling the separation
of peptides that would otherwise
co-elute.36,39,40
In standard MS/MS experiments, large
amounts of ions are discarded, as only a
small proportion of the ion beam is selected
for analysis. However, in PASEF, storage
in the TIMS cell and rapid quadrupole
switching times enable the selection and
identification of a far wider range of precur-
MS-based proteomics approaches are rapidly
gaining traction in the TPD field for analysis
of on- and off-target effects. These methods
can significantly increase the efficiency of
optimizing TPD therapeutics
Targeted Protein Degraders 7
What is TPD?
sors, resulting in up to a 10-fold increase in
sequencing speed and increased sensitivity.40
The combination of PASEF with data-independent
acquisition methods (dia-PASEF)
gives a quantitative result for every analyte in
a sample. This reduces the risk that the same
precursors won’t be selected in multiple
samples and ensures that identification and
quantitation results are highly reproducible
between samples, even in large cohorts.34
A complete proteomics workflow that
incorporates TIMS and dia-PASEF results
in a range of potential advantages for TPD
development. High sensitivity coupled with
the reduced co-fragmentation rates offered by
TIMS and dia-PASEF can decrease spectral
complexity and contribute to higher and more
accurate identification rates than traditional
methods.41 The increased speed and high
efficiency of PASEF technology makes it the
ideal method for deep proteome profiling
and quantitation, with previous experiments
showing successful profiling of at least 50
to 100 human cell lysate samples per day.42
Overall, this workflow offers an unparalleled
insight into the on- and off-target effects of
targeted protein degraders and promises to
put an end to the “undruggable” proteome.
Revolutionize your
TPD workflow today
References
1. Precedence Research. 2022. “Drug
Discovery Market - Global Industry Analysis,
Size, Share, Growth, Trends, Regional
Outlook, and Forecast 2023 – 2032.”
Accessed June 15, 2023. https://www.
precedenceresearch.com/drug-discoverymarket.
2. Craven, Jeff. 2023. “CDER Approved 37
Novel Drugs in 2022, Its Lowest Number
since 2016.” RAPS. Accessed June 14,
2023. https://www.raps.org/news-andarticles/
news-articles/2023/1/cder-approves-
37-novel-drugs-in-2022-its-lowest-nu.
3. Institute of Medicine. 2014. “Improving
and Accelerating Therapeutic Development
for Nervous System Disorders: Workshop
Summary. Washington, DC: The National
Academies Press. doi:10.17226/18494.
4. Coleman, Niamh, and Jordi Rodon. 2021.
“Taking Aim at the Undruggable.” American
Society of Clinical Oncology Educational
Book, 41. doi:10.1200/edbk_325885.
5. Spradlin, Jessica N., Erika Zhang, and
Daniel K. Nomura.
2021. “Reimagining
Druggability Using Chemoproteomic
Platforms.” Accounts of Chemical
Research 54 (7): 1801–13. doi:10.1021/acs.
accounts.1c00065.
6. Chamberlain, Philip P., and Lawrence G.
Hamann. 2019. “Development of Targeted
Protein Degradation Therapeutics.”
Nature Chemical Biology 15 (10): 937–44.
doi:10.1038/s41589-019-0362-y.
7. Zhao, Lin, Jia Zhao, Kunhong Zhong, Aiping
Tong, and Da Jia. 2022. “Targeted Protein
Degradation: Mechanisms,
Strategies
and Application.” Signal Transduction
and
Targeted Therapy 7 (113). doi:10.1038/
s41392-022-00966-4.
8. Cooper, Geoffrey M., and Robert E. Hausman.
2000. The Cell; a Molecular Approach.
Oxford: Sinauer Associates. Available
from: https://www.ncbi.nlm.nih.gov/books/
NBK9839/.
9. Tai, Hwan-Ching, and Erin M. Schuman.
2008. “Ubiquitin,
the Proteasome and
Protein Degradation in Neuronal Function
and Dysfunction.” Nature Reviews
Neuroscience 9 (11): 826–38. doi:10.1038/
nrn2499.
10. Park, E.C., D. Finley, and J.W. Szostak. 1992.
“A Strategy
for the Generation of Conditional
Mutations by Protein Destabilization.”
Proceedings of the National Academy of
Sciences 89 (4): 1249–52. doi:10.1073/
pnas.89.4.1249.
11. Natsume, Toyoaki, and Masato T. Kanemaki.
2017. “Conditional Degrons for Controlling
Protein Expression at the Protein Level.”
Annual Review of Genetics 51 (1): 83–102.
doi:10.1146/annurev-genet-120116-024656.
12. Paiva, Stacey-Lynn, and Craig M. Crews.
2019. “Targeted Protein Degradation:
Elements of PROTAC Design.” Current
Opinion in Chemical Biology 50: 111–19.
doi:10.1016/j.cbpa.2019.02.022.
13. Rana, Sandeep, Jayapal Reddy Mallareddy,
Sarbjit Singh, Lidia Boghean, and Amarnath
Natarajan. 2021. “Inhibitors, PROTACs and
Molecular Glues as Diverse Therapeutic
Modalities to Target Cyclin-Dependent
Kinase.” Cancers 13 (21): 5506. doi:10.3390/
cancers13215506.
14. Békés, Miklós, David R. Langley, and Craig
M. Crews. 2022. “PROTAC Targeted Protein
Degraders: The Past Is Prologue.” Nature
Reviews Drug Discovery 21 (3): 181–200.
doi:10.1038/s41573-021-00371-6.
15. Petrylak, Daniel Peter, Xin Gao, Nicholas
J. Vogelzang, Mary Harlow Garfield, Ian
Taylor, Marcia Dougan Moore, Ronald Alan
Peck, and Howard A. Burris. 2020. “Firstin-
Human Phase I Study of ARV-110, an
Androgen Receptor (AR) PROTAC Degrader
in Patients
(PTS) with Metastatic Castrate-
Resistant Prostate
Cancer (Mcrpc) Following
Enzalutamide (ENZ) and/or Abiraterone
(ABI).” Journal of Clinical Oncology 38
(15): 3500. doi:10.1200/jco.2020.38.15_
suppl.3500.
16. Chirnomas, Deborah, Keith R. Hornberger,
and Craig M. Crews. 2023. “Protein Degraders
Enter the Clinic — a New Approach to
Cancer Therapy.” Nature Reviews Clinical
Oncology 20 (4): 265–78. doi:10.1038/
s41571-023-00736-3.
17. Clague, Michael J., Claire Heride, and Sylvie
Urbé. 2015. “The Demographics of the
Ubiquitin
System.” Trends in Cell Biology 25
(7): 417–26. doi:10.1016/j.tcb.2015.03.002.
18. Schapira, Matthieu, Matthew F. Calabrese,
Alex N. Bullock, and Craig M. Crews. 2019.
“Targeted Protein Degradation: Expanding
the Toolbox.” Nature Reviews Drug
Discovery 18 (12): 949–63. doi:10.1038/
s41573-019-0047-y.
19. Chamberlain, Philip P., and Brian E. Cathers.
2019. “Cereblon Modulators: Low Molecular
Weight Inducers of Protein Degradation.”
Drug Discovery Today: Technologies 31:
29–34. doi:10.1016/j.ddtec.2019.02.004.
20. Zheng, Shuangjia, Youhai Tan, Zhenyu Wang,
Chengtao
Li, Zhiqing Zhang, Xu Sang,
Hongming Chen, and Yuedong Yang. 2022.
“Accelerated Rational PROTAC Design via
Deep Learning and Molecular Simulations.”
Nature Machine Intelligence 4 (9): 739–48.
doi:10.1038/s42256-022-00527-y.
21. Edmondson, Scott D., Bin Yang, and
Charlene Fallan. 2019. “Proteolysis Targeting
Chimeras (Protacs) in ‘beyond Rule-of-Five’
Chemical Space: Recent Progress and
Future Challenges.” Bioorganic & Medicinal
Chemistry
Letters 29 (13): 1555–64.
doi:10.1016/j.bmcl.2019.04.030.
22. Kostic, Milka, and Lyn H. Jones. 2020.
“Critical Assessment of Targeted Protein
Degradation as a Research Tool and Pharmacological
Modality.” Trends in PharmacologTargeted
Protein Degraders 8
What is TPD?
ical Sciences 41 (5): 305–17. doi:10.1016/j.
tips.2020.02.006.
23. Tolvanen, Tuomas Aleksi. 2022. “Current Advances
in CETSA.” Frontiers in Molecular Biosciences 9.
doi:10.3389/fmolb.2022.866764.
24. Seashore-Ludlow, Brinton, Hanna Axelsson,
and Thomas Lundbäck. 2020. “Perspective on
CETSA Literature: Toward More Quantitative Data
Interpretation.” SLAS Discovery 25 (2): 118–26.
doi:10.1177/2472555219884524.
25. Sanchez, Tino W., Michael H. Ronzetti, Ashley E.
Owens, Maria Antony, Ty Voss, Eric Wallgren, Daniel
Talley, et al. 2022. Real-Time Cellular Thermal Shift
Assay (RT-CETSA) to Monitor Target Engagement.
ACS Chemical Biology 17 (9): 2471–82. doi:10.1021/
acschembio.2c00334.
26. Liu, Xingui, Xuan Zhang, Dongwen Lv, Yaxia Yuan,
Guangrong Zheng, and Daohong Zhou. 2020. “Assays
and Technologies for Developing Proteolysis Targeting
Chimera Degraders.” Future Medicinal Chemistry 12
(12). doi:10.4155/fmc-2020-0073.
27. Riching, Kristin M., Sarah Mahan, Cesear R. Corona,
Mark McDougall, James D. Vasta, Matthew B.
Robers, Marjeta Urh, and Danette L. Daniels. 2018.
“Quantitative Live-Cell Kinetic Degradation and
Mechanistic Profiling of PROTAC Mode of Action.”
ACS Chemical Biology 13 (9): 2758–70. doi:10.1021/
acschembio.8b00692.
28. Pettersson, Mariell, and Craig M. Crews. 2019. “Proteolysis
Targeting Chimeras (PROTACs) — Past, Present
and Future.” Drug Discovery Today: Technologies 31:
15–27. doi:10.1016/j.ddtec.2019.01.002.
29. Bondeson, Daniel P., Blake E. Smith, George M.
Burslem, Alexandru D. Buhimschi, John Hines, Saul
Jaime-Figueroa, Jing Wang, Brian D. Hamman, Alexey
Ishchenko, and Craig M. Crews. 2018. “Lessons in
PROTAC Design from Selective Degradation with a
Promiscuous
Warhead.” Cell Chemical Biology 25 (1):
78–87. doi:10.1016/j.chembiol.2017.09.010.
30. Liu, Xin, Ye Zhang, Lucas D. Ward, Qinghong Yan,
Tanggis Bohnuud, Rocio Hernandez, Socheata
Lao, Jing Yuan, and Fan Fan. 2021. “A Proteomic
Platform to Identify Off-Target Proteins Associated
with Therapeutic Modalities That Induce Protein
Degradation or Gene Silencing.” Scientific Reports 11
(1). doi:10.1038/s41598-021-95354-3.
31. Chernobrovkin, Alexey L., Cindy Cázares-
Körner,
Tomas Friman, Isabel Martin Caballero, Daniele
Amadio, and Daniel Martinez Molina. 2021. “A Tale
of Two Tails: Efficient Profiling of Protein Degraders
by Specific Functional and Target Engagement
Readouts.” SLAS Discovery 26 (4): 534–46.
doi:10.1177/2472555220984372.
32. Grohmann, Christoph, Charlene M. Magtoto, Joel
R. Walker, Ngee Kiat Chua, Anna Gabrielyan,
Mary
Hall, Simon A. Cobbold, et al. 2022. “Development of
NanoLuc-Targeting Protein Degraders and a Universal
Reporter System to Benchmark Tag-Targeted
Degradation
Platforms.” Nature Communications 13
(1). doi:10.1038/s41467-022-29670-1.
33. Zhao, Jianyuan, Jing Wang, Xu Pang, Zhenlong Liu,
Quanjie Li, Dongrong Yi, Yongxin Zhang, et al. 2022.
“An Anti-Influenza A Virus Microbial Metabolite Acts
by Degrading Viral Endonuclease PA.” Nature Communications
13 (1). doi:10.1038/s41467-022-29690-x.
34. Kruppa, Gary. 2021. “Dia-PASEF: A Result of the
Co-Evolution of Mass Spectrometry and Proteomics.”
Labmate Online. Accessed June 15, 2023. https://
www.labmate-online.com/article/mass-spectrometryand-
spectroscopy/41/bruker-daltonics/dia-pasef-aresult-
of-the-co-evolution-of-mass-spectrometry-
andproteomics/
2910.
35. Aballo, Timothy J., David S. Roberts, Jake A. Melby,
Kevin M. Buck, Kyle A. Brown, and Ying Ge. 2021.
“Ultrafast and Reproducible Proteomics from
Small Amounts of Heart Tissue Enabled by Azo and
TIMSTOF Pro.” Journal of Proteome Research 20 (8):
4203– 11. doi:10.1021/acs.jproteome.1c00446.
36. Meier, Florian, Melvin A. Park, and Matthias Mann.
2021. “Trapped Ion Mobility Spectrometry and Parallel
Accumulation–Serial Fragmentation in Proteomics.”
Molecular & Cellular Proteomics 20: 100138.
doi:10.1016/j.mcpro.2021.100138.
37. Ridgeway, Mark E., Markus Lubeck, Jan Jordens,
Mattias Mann, and Melvin A. Park. 2018. “Trapped
Ion Mobility Spectrometry: A Short Review.” International
Journal of Mass Spectrometry 425: 22–35.
doi:10.1016/j.ijms.2018.01.006.
38. Tose, Lilian V., Paolo Benigni, Dennys Leyva, Abigail
Sundberg, César E. Ramírez, Mark E. Ridgeway,
Melvin A. Park, Wanderson Romão, Rudolf Jaffé, and
Francisco Fernandez-Lima. 2018. “Coupling Trapped
Ion Mobility Spectrometry to Mass Spectrometry:
Trapped Ion Mobility Spectrometry–Time-of-flight
Mass Spectrometry versus Trapped Ion Mobility
Spectrometry–Fourier Transform Ion Cyclotron Resonance
Mass Spectrometry.” Rapid Communications
in Mass Spectrometry 32 (15): 1287–95. doi:10.1002/
rcm.8165.
39. Demichev, Vadim, Lukasz Szyrwiel, Fengchao Yu,
Guo Ci Teo, George Rosenberger, Agathe Niewienda,
Daniela Ludwig, et al. 2022. “Dia-PASEF Data
Analysis Using FragPipe and Dia-NN for Deep
Proteomics of Low Sample Amounts.” Nature
Communications 13 (1). doi:10.1038/s41467-022-
31492-0.
40. Meier, Florian, Scarlet Beck, Niklas Grassl, Markus
Lubeck, Melvin A. Park, Oliver Raether, and Matthias
Mann. 2015. “Parallel Accumulation–Serial Fragmentation
(PASEF): Multiplying Sequencing Speed and
Sensitivity by Synchronized Scans in a Trapped Ion
Mobility Device.” Journal of Proteome Research 14
(12): 5378–87. doi:10.1021/acs.jproteome.5b00932.
41. Charkow, Joshua, and Hannes L. Röst. 2021.
“Trapped Ion Mobility Spectrometry Reduces
Spectral Complexity in Mass Spectrometry-Based
Proteomics.” Analytical Chemistry 93 (50): 16751–58.
doi:10.1021/acs.analchem.1c01399.
42. Hebeler, Romano, Stephanie Kaspar-
Schoenefeld,
and Gary Kruppa. 2022. “Speeding up Label-Free
Quantitation of Complex Proteome Samples Using
Dia-PASEF.” Bruker Daltonics. Accessed June 15,
2023. https://www.bruker.com/en/products-andsolutions/
mass-spectrometry/timstof/timstof-ht/_jcr_
content/root/sections/more_information/sectionpar/
search.download-asset.pdf/8ec1ea93-0ac8-4c5db408-
bd7c0ce1fe34/1803363-lcms-201-dia-pasef-lfqebook.
pdf.
Targeted Protein Degraders 9
What is TPD?
Targeted Protein
Degradation
The Future of Drug Development
Despite continuous advances in the drug development
industry, around 85% of the human proteome is still
considered “undruggable”.1
Targeted protein degradation (TPD) is rapidly gaining
traction as a new therapeutic strategy to target formally
undruggable proteins. However, only a small number
of degraders have been explored in clinical trials.
This infographic discusses the main challenges in
TPD workflows and presents innovative solutions to
accelerate drug development.
A complete solution for TPD analysis
From PreOmics sample preparation kits to the timsTOF HT and choices of data processing software,
Bruker offers a range of solutions to support your TPD quantitative proteomics workflow at every stage.
Bruker PepSep™ Columns
• Robust, high performance nanoLC columns
timsTOF HT
• Uniquely enables rapid dia-PASEF experiments,
with unparalleled depth of coverage and
quantitation in large cohorts
Bruker ProteoScape™
• Real-time data processing with TIMS DIA-NN
Spectronaut® by Biognosys
• Comprehensive peptide identification with
industry standard
REFERENCES
1. Spradlin, Jessica N., Erika Zhang, and Daniel K. Nomura. “Reimagining Druggability Using Chemoproteomic Platforms.” Accounts of Chemical Research 54, no. 7
(2021): 1801–13. https://doi.org/10.1021/acs.accounts.1c00065.
Discover the future of TPD drug development.
Target
Proteasome
Target
ligand
E3
ligand
E3 ligase
Linker
E2
E2
E2
E2
E2
Target
Molecular
glue E3 ligase
E2
Proteasome
What is TPD?
In TPD, small molecule ligands such as PROTACs and molecular glues are designed to link a specific
protein of interest (POI) to an E3 ligase. This redirects the POI towards the cellular degradation machinery.
How are targeted protein degraders developed?
Most degraders are discovered through the screening of vast molecular libraries, in a complex, multi-step
process. Therefore, the need for rapid high-throughput capabilities is paramount in order to not only screen
these large libraries, but to attain statistically relevant data and confidently select promising candidates.
Pushing the boundaries of degrader development
Parallel accumulation serial fragmentation (PASEF) enabled by trapped ion mobility spectrometry (TIMS) improves
sequencing speed, accuracy and sensitivity by accumulating and then separating ions in the gas-phase. The Collisional
Cross Section (CCS) of the molecule, an inherent physical property, is used to separate isobaric peptides, improving the
accuracy of identification.
When coupled with time of flight (TOF) mass spectrometry and PASEF, these methods provide unparalleled
confidence and accuracy in your protein identification and quantitation.
Increases selectivity
for complex samples
in short run times
Increases sensitivity
by reducing
background noise
Increases run speed,
enabling highthroughput
Increases accuracy
for highly confident
identification
Enables deep
sequencing methods
for comprehensive
proteome analysis
80
60
40
20
0
-4 -2 0 2 4
Fold change log
Significance
80
60
40
20
0
-4 -2 0 2 4
Fold change log
Significance
See the bigger picture with high throughput proteomics
Assessing off-target effects is crucial for drug development. Failure to address these early on
can result in high levels of attrition due to safety issues. While end-point assays can produce
accurate data, they often lack the scope to assess off-target effects. Proteomic approaches
give a complete picture of TPD effects in the cell during novel degrader screens.
End-point assays show specific data
about the protein of interest.
Proteomics approaches show change
in expression for the whole proteome.
• Hypothesis driven
• Time-consuming
• Can be low-throughput
• Rapidly examines changes in abundance for
thousands of proteins
• Identifies on- and off-target effects
• Supports characterization of mechanism of
action
1 2 3 4 5 6
POI POI
Off-target
effect
Other
cellular
proteins
!
Select target
protein and
ligand
Design and
synthesize
degrader
libraries
Assess target
engagement and
degradation
e.g., immunoblotting,
fluorescence, mass
spectrometry
Analyse
ternary complex
formation
e.g., in silico methods,
co-crystal structure,
surface plasmon
resonance, native mass
spectrometry
Investigate
selectivity and
off-target effects
e.g., mass
spectrometry-based
proteomics
Further
evaluation
e.g.,
pharmacokinectics,
in vivo testing
Retention time Mass-to-charge (m/z) ratio
Intensity Collisional Cross
Section (shape)
Molecular properties
measured
Requires a high-throughput, proteomewide
readout in order to accurately assess
cell-wide effects.
PreOmics iST
Technology
• Versatile and
simple: All-in-one
and ready-touse
proteomics
sample
preparation kits
for a variety of
applications.
• Fast: The kits only
require 1 hour of
hands-on time,
with a total time
of less than 2.5
hours.
!
Degrader design
Constructed to bind both
target and E3 ligase, either
through a linker (PROTAC)
or directly (molecular glue)
1
Ternary complex
Degrader recruits target to
E3 ligase
2
Polyubiquitination
Ubiquitin (Ub) added to
target’s Lys residues
3
Target degradation
Ub-marked target
is degraded by the
proteasome
4
Developing novel TPD drug candidates is
a complex process owing to the lack of
easily druggable pockets on the protein of
interest. Mass spectrometry (MS)-based
proteomics is essential in revealing the
complete target spectrum of degrader
molecules, confirming on-target degradation,
highlighting off-target regulation and
identifying potential novel targets. Here,
high throughput and rapid turnaround are
key to accelerating drug development and
avoiding significant bottlenecks. NEOsphere
Biotechnologies specializes in deep proteomic
screening and MS-based mechanistic
validation of potential novel targets
to advance the development of degrader
drugs for previously undruggable targets.
This interview explores the technology of
NEOsphere Biotechnology and its impacts
on TPD drug discovery.
NEOsphere Biotechnologies:
Breaking the Barriers of TPD
Drug Discovery
Dr. Jutta Fritz
CBO and co-founder
NEOsphere Biotechnologies
Dr. Fritz is a business development expert with more than
15 years of management experience within the life sciences
and diagnostics industries. Prior to joining NEOsphere
Biotechnologies, she was co-founder and CBO of the cancer
diagnostics company NEO New Oncology, VP of Business
Development for Proteomics Services at Evotec and Head of
Business Development at the proteomics company Kinaxo
Biotechnologies. Dr. Fritz has a PhD in molecular biology from
the University of Vienna and an MBA in financial management
from the University of Wales.
Dr. Uli Ohmayer
Head of Mass Spectrometry and co-founder
NEOsphere Biotechnologies
Dr. Ohmayer is a leading expert in industrial-scale, deep
proteomic screening. He has over a decade of experience
in MS-based proteomics and broad expertise in laboratory
automation and rapid scaling of proteomics infrastructure. In
his previous position at Evotec, he was instrumental in the
development of data-independent acquisition MS for singleshot
proteomics with unprecedented throughput, depth and
sensitivity. Dr. Ohmayer has a PhD in biochemistry from the
University of Regensburg and was a postdoctoral researcher
in the Mass Spectrometry Core Unit of Helmholtz Zentrum
Munich.
››
››
Targeted Protein Degraders 11
Ask the Experts
Q: Can you give us a brief overview
of NEOsphere Biotechnologies? What
sets you apart from other companies
in the field?
Jutta Fritz (JF): NEOsphere Biotechnologies
is a leader in the field of MS-based
proteomics for drug discovery, with a focus
on targeted protein degradation. Founded in
2022 and located in Munich, we work with
pharmaceutical and biotechnology companies
to systematically evaluate the true target
scope of their degrader compounds in a proteome-
wide context.
Our technology combines the highest data
quality and proteome coverage with high
throughput and fast turnaround time, making
it ideal for supporting drug discovery and
optimization. To this end, all our laboratory
and data analysis processes are automated
and scalable.
Our deep proteomic screening platform
reveals changes in protein regulation upon
compound treatment. The analysis is performed
on intact, unmodified cells to monitor
compound selectivity in endogenous environments.
We routinely quantify up to 11,000
proteins in a single experiment, allowing us to
comprehensively evaluate degrader efficacy,
assess off-target effects and identify potential
new target proteins for degraders. In this
regard, our deep proteome coverage combined
with reliable protein quantification is
critical for the identification of low-abundance
proteins that may be of great interest for
drug discovery, such as transcription factors.
It is also possible to analyze the effect of a
degrader on the whole proteome at different
time points or concentrations to determine
how quickly and strongly it acts and at what
point secondary effects may occur. Thanks
to our high-throughput capabilities, we can
screen degrader libraries of thousands of
compounds (for an example of how deep
proteomic screening data is presented, see
Figure 1).
In addition to deep proteomic screening, we
offer MS-based technologies to mechanistically
validate potential degrader targets, e.g.,
by ubiquitinomics or high throughput interactomics.
In this way, potentially interesting
hits from the deep proteomic screen can be
further investigated immediately.
Figure 1. Comprehensive detection of compound-specific and cell line-specific degradation events. HCT-116 cells were treated with two immunomodulatory
imides (IMiDs) and one PROTAC as indicated (upper panel) and analyzed by single-shot MS analysis. The volcano plots illustrate significant up- (in blue) and
downregulations (in red) induced by the different compounds. The x-axis depicts the fold change (log2) of proteins in compound vs DMSO-treated cells and
the y-axis depicts the standard error. Known cellular targets such as zinc finger proteins were detected for pomalidomide and mezigdomide. SMARCA2 and
SMARCA4 were downregulated upon 4 hours treatment with the VHL based PROTAC ACBI1, together with two interacting BAF complex members, while
secondary regulation was seen at a later 8 hours time point. Additional cell lines were treated with pomalidomide for 5 hours (lower panel) and compared to
HCT-116. ZFP91 was significantly down-regulated in all cell lines, other neosubstrates exhibited varying degrees of regulation, reflecting cell type-specific
expression (e.g., IKZF1 in U937 and NB-4, or SALL4 in SUSA) or different levels of IMiD responsiveness to commonly expressed neosubstrates. In total, 17
different neosubstrates were significantly downregulated in at least one cell line.
Targeted Protein Degraders 12
Ask the Experts
Q: What is your process for screening
potential TPD drug candidates?
Uli Ohmayer (UO): To evaluate and quantify
the effect of a compound on protein degradation
while maintaining very short turnaround
times, we have developed scalable and
robust laboratory processes and statistical
methods. We routinely work in a 96-well
plate format and test every compound in triplicate,
which enables very powerful statistical
analyses. Like compound treatment, sample
preparation is largely automated. First, we
perform a tryptic digest followed by peptide
purification so that the complete cellular
proteome is cleaved into peptides, which are
then separated by liquid chromatography (LC)
and analyzed by MS.
To minimize technical variation, maximize
data completeness and significantly reduce
measurement time for mass spectrometry,
we don’t use chemical labeling followed by
off-line fractionation, but measure samples
in a label-free single-shot approach. For MS
analysis, we use dia-PASEF (data-independent
acquisition and parallel accumulation and
serial fragmentation) on timsTOF instruments
from Bruker. Here, ion mobility separation
is used to reduce signal interference
and increase the sensitivity of proteomics
analysis. This results in very deep proteome
coverage and detection of more than 200,000
precursor ions in one sample, corresponding
to approximately 11,000 proteins. Each mass
spectrometry run generates a large amount
of highly complex raw data. We use DIA-NN
software developed by one of our scientific
advisors, Dr. Vadim Demichev, as well as
proprietary data analysis and statistical tools
developed at NEOsphere for the analysis. To
detect statistically significant protein regulation
upon compound treatment, a comparison
is made with untreated controls.
To determine whether regulation is due to
protein degradation or other effects, we can
then perform mechanistic validation of all
potential hits identified in the screen using
additional MS-based tools such as interactomics
or ubiquitinomics. Degrader compounds
induce proximity between the E3 ligase and
the protein of interest (POI) and initiate ubiquitination
of the POI, marking it for degradation
by the proteasome. Our ubiquitination
assay quantifies up to 50,000 ubiquitination
sites in a single experiment. Comparing the
regulation of ubiquitination sites in treated
and untreated cells provides clear clues as to
whether the protein regulation observed in
the proteomic screen is indeed due to degradation.
NEOsphere’s ubiquitinomics platform
stands out in the field for its depth, speed,
precision and throughput.
Q: How do you optimize the assays
in your pipeline to ensure maximum
efficiency?
OU: We have systematically tested and
optimized a variety of parameters for all the
Figure 2. Combined analysis of proteomics and ubiquitinomics reveals and validates primary degrader targets. HEK293 cells were treated with the cereblon
modulator avadomide. Ubiquitinomics was performed using MS-based K-GG remnant profiling, enabling the quantification of up to 50,000 ubiquitination sites
in single DIA-MS runs without cellular proteasome inhibition. Ubiquitinomics revealed induced ubiquitination sites for almost all neosubstrates found to be
degraded upon 5 hrs treatment of HEK293 cells. These included sites on IKZF3, a protein not detected in the proteomics experiment due to its extremely low
expression in HEK293 cells. Ubiquitinomics allows the rapid validation of cellular downregulations due to E3 ligase-neosubstrate relationships, by analyzing
degrader drugs in endogenous cellular systems without the need for pharmacological intervention or genetic modification.
Targeted Protein Degraders 13
Ask the Experts
steps of our workflow, from cell treatment
and sample preparation to data acquisition,
data analysis and statistical evaluation. We
have also adjusted all instrument settings to
simultaneously achieve very deep proteome
coverage and precise protein quantification.
At each stage there are many details that can
be optimized, and although each of these
individual changes may have a rather small
effect, they multiply and eventually lead to a
highly efficient process.
JF: Continuous optimization is very important
– our workflows are state-of-the-art, but it’s
a constant process to maintain them as such
and to keep pushing the technological boundaries.
All our processes are designed to best
support the requirements of drug discovery.
We can analyze all types of degraders such
as molecular glues, PROTACs, DUB-inhibitors
and monovalent degraders. The technology
can also be used to measure the effect of protein
stabilizers on the proteome. In terms of
material, we are very flexible and can use, for
example, adhesive and suspension cell lines
or primary cells.
Q: How do you validate your results?
What kind of measures do you take
to ensure that they are reliable and
reproducible?
UO: Our technology is quite sophisticated
and complex, so strict quality control must be
performed on every sample. For example, we
continuously check parameters such as digestion
efficiency, mass accuracy or peak widths
in near real-time to ensure that the performance
is always at the required level. By
testing samples in triplicate, we can calculate
a coefficient of variation and determine reproducibility
and consistency between replicates.
Our automated data processing includes
numerous stringent control mechanisms to
ensure the highest data quality. For example,
filtering is applied to further enhance data
completeness and allow precise quantification
of even low abundance proteins, and
numerous biostatistical tests are routinely
performed to improve statistical power.
Q: What are some of the biggest
challenges you face when screening
degraders and how do you overcome
these challenges?
UO: Apart from turnaround time, one of
the biggest challenges in deep proteomic
screening is throughput, especially when
testing large libraries of tens of thousands of
compounds. Screening therefore requires a
platform that can measure multiple samples
in parallel while keeping the time to analyze a
set of samples as short as possible. To meet
this requirement, we have built our platform so
that each step is scalable. If a project requires
screening of large number of compounds, we
can thus meet that demand very quickly.
Q: How do you see the field of TPD
evolving over the next few years?
What role do you think MS will play in
shaping the future of this industry?
UO: The structure–activity relationship is very
steep particularly for molecular glues – even
changes by one atom can cause significant
changes in compound potency and cellular
target selectivity. MS is the key technology to
identify these changes and potential off-target
effects. The proteomic data we generate is
also very useful for developing the chemistry
of degrader drug candidates. Chemists can
use our data to further optimize the chemical
structure of the degraders and implement a
more rational approach to degrader design,
making drug discovery much faster and more
reliable than current methods.
JF: Targeted protein degradation holds enormous
potential to address many urgent clinical
needs. It’s still a young but rapidly growing
field, with several promising compounds
currently in clinical trials. In our opinion,
MS-based proteomics will become one of
the key factors for successful development of
degrader compounds and thus will gain much
importance in the future.
NEOsphere Biotechnologies
Proteomic screening to unlock the potential of protein degraders
Watch now
Targeted Protein Degraders 14
Ask the Experts
Current drug discovery processes have barely
scratched the surface of targeted protein
degradation. Only a handful of E3 ligases
have been clinically evaluated until now, and
many discovery processes have relied on
the serendipitous identification of degraders.
To discover the full potential of TPD for the
undruggable proteome, high-throughput,
rational design approaches are required.
Plexium uses a comprehensive platform to
develop monovalent protein degraders across
a range of modalities. This interview explores
Plexium’s technologies and their potential to
develop next-generation TPD drugs.
Q: Can you give us a brief overview
of Plexium? What sets you apart from
other companies in the field?
Alex Campos (AC): Plexium is the premier,
next-generation targeted protein degradation
(TPD) company focused on pursuing a new
class of selective TPD drugs called “direct
degraders”. These are small molecules
designed to bind to a pathogenic protein to
induce selective degradation of the protein by
the cell’s natural protein quality control machinery.
In addition to direct degraders, Plexium
is pursuing molecular glues, an approach
that also leverages the cell’s natural quality
control machinery. However, in this case, small
molecules bind to an E3 ligase, then redirect
the ligase to selectively engage and degrade
pathogenic proteins for degradation.
Our company has developed a comprehensive
approach toward TPD which is powered
by our proprietary best-in-class platform that
enables us to discover a wide variety of TPD
modalities, from molecular glues to monovalent
degraders, while also identifying novel E3
ligases beyond cereblon and the von Hippel–
Lindau complex (VHL). We are working toward
the discovery of next-generation TPD drugs
across multiple therapeutic areas.
As a senior director in the drug discovery
department, my role is to lead the proteomics
platform, overseeing projects, operations and
technologies within the proteomics realm. In
addition, I’m actively involved in proteomics
data processing, analysis and interpretation to
help downstream project decisions. Given the
highly collaborative and innovative nature of
Plexium as an organization, I work closely with
other drug discovery team leaders to further
optimize our platform and deliver cutting-edge
technologies for TPD.
Plexium’s Next-Generation TPD
Dr. Alex Campos
Head of Proteomics
Plexium
Alex Campos joined Plexium in 2022 as senior director in
the drug discovery department and head of the proteomics
department, bringing more than 20 years of experience in
proteomics and data science. Prior to Plexium, Alex was at
Sanford-Burnham-Prebys (SBP) Medical Discovery Institute
where he conducted his postdoc in proteomics as part of a
collaboration between SBP and MedImmune and continued on
into varying roles of increasing responsibility in the proteomics
core. From 2016–2021, Alex served as director of the
proteomics core and member of the NCI-designated Cancer
Center and initiated important collaborations within emerging
TPD companies from San Diego, Boston and San Francisco.
He received his PhD in molecular pathology with a focus in
proteomics technology in biomedicine from the University of
Barcelona where he worked at the Barcelona Science Park.
››
Targeted Protein Degraders 15
Ask the Experts
Q: What is your process for designing
targeted protein degraders? How
do you identify potential targets and
design degraders specific to those
targets?
AC: Plexium is pursuing drug targets that have
previously been undruggable or inadequately
drugged within the oncology and neuroscience
disease areas. We believe that we have a rich
and diverse portfolio including known valuable
targets such as IKZF2 and SMARCA2, among
other important cancer-related proteins.
The company is powered by its proprietary
TPD drug discovery platform. This integrates
degrader chemistry design principles and
screening libraries with cell-based, target-specific
degradation assays, including an ultrahigh-
throughput screening platform, to enable
the identification of drug-like, cell-permeable
degrader molecules. This integrated TPD drug
discovery approach has led to the discovery
of novel, selective direct degraders, molecular
glue drug candidates and two unprecedented
E3 ligases.
Plexium has established validated chemistry
design principles for target-selective protein
degraders and deploys multiple chemistry
approaches to generate molecules for
medicinal chemistry optimization. Bespoke
libraries are constructed for each target. Once
target binders are identified, structure-based,
ligand-based and diversity-based approaches
are deployed for library design within a druglike
space. Our DNA-encoded “one-bead,
one-compound” libraries enable the sampling
of diverse chemical space. The compounds are
tethered to a bead with a photocleavable linker.
The bead is then transferred to an assay device
well and ultraviolet light is utilized to release
the compounds from the bead. Our novel
approach overcomes drug screening limitations
associated with traditional DNA-encoded
libraries and enables cell-based screening of
DNA-encoded libraries in a one compound per
well configuration.
Q: How do you screen TPD candidates
and evaluate their efficacy in vivo? How
do you optimize these assays for maximum
efficiency?
AC: Plexium’s proprietary miniaturized assay
devices contain up to 88,000 isolated screening
wells in a device with a footprint similar to
a conventional 96-well plate device, with 20
to 50 cells per well. On-bead libraries enable
single compound per well assays. Plexium’s
tabletop cell-based μHTS instrument supports
high content, multiplexed assays. Our assay
endpoint is the degradation of one or more
proteins of interest in a disease representative
cell. Upon assay completion, images are
acquired and analyzed to support “hit” calling.
The beads associated with hit compounds contain
a DNA barcode that uniquely identifies the
single compound on that bead, which can be
read for compound identification. At this stage,
we start a cascade of assays to validate our
cellularly-active degrader hits using mainly cell
models relevant to the target of interest.
Medicinal chemistry optimization of degradation,
potency, selectivity and ADME properties
is an important part of this process. In
addition, our Biology department (in particular
the in-vivo pharmacology team), will conduct
good laboratory practice (GLP)-toxicology/
pharmacology studies and early human clinical
trials to evaluate response and safety of lead
compounds. Importantly, we identify biomarkers
to guide patient selection for human clinical
trials as well.
Q: How do you validate your results?
What measures do you take to ensure
that they are reliable and reproducible?
AC: We rely on different techniques to validate
our screening results. Target engagement
is usually confirmed with HiBiT or surface
plasmon resonance (SPR). Degradation of the
target and dependency of the 26S proteasome
is commonly confirmed with Western blots
with or without co-treatment of cells with neddylation
or proteasome inhibitors. However,
mass spectrometry (MS) quantitative proteomics
is the ultimate technique to evaluate onand
off-target degradation across different cell
lines and compound concentrations. At this
point, we can also use neddylation or proteasome
inhibitors to evaluate the proteasome-dependency
of the protein degradation.
Reproducibility and confidence are important
aspects of our work. Compliance to standard
operating procedures is crucial to obtain
reliable results. In addition, robust data analysis
processes and proper experimental design
are essential; for example, using controls and
running replicates for the different tested conditions
helps to power our analysis, particularly
for proteomics technologies.
Targeted Protein Degraders 16
Ask the Experts
Q: What are some of the biggest
challenges you face when developing
and screening TPDs, and how do you
overcome these challenges?
AC: A deep understanding of the target protein
is crucial for successful screening campaigns.
Protein-level information on target expression
distribution in tissues/cell lines, protein turnover,
protein-protein interactions and subcellular
compartment localization help us to design
the screening conditions and assay cascade.
Despite the large volume of genomic data in
public repositories, we still lack a thorough protein-
level database. For example, protein-level
data in TCGA is very sparse compared to
genomics data. At Plexium, we use MS proteomics
to map proteins in cells and tissues
and create a cell-specific atlas of the proteome
to help design successful TPD screenings.
There are also several challenges associated
with the chemistry of designing and screening
monovalent degraders. The cellular degradation
machinery may have unintended effects
on non-target proteins, leading to off-target
toxicity. Designing ligands with high selectivity
and minimizing off-target effects is crucial
to ensure the safety and efficacy of targeted
protein degradation therapies. In addition,
designing ligands with optimal properties, such
as appropriate binding affinity, selectivity and
cell permeability, is a complex task. To date,
most monovalent degraders have been found
serendipitously, and there are no clear rational
chemical design principles for converting protein-
targeting ligands into monovalent degraders.
However, Plexium has already established
validated chemistry design principles for target
selective protein degraders. The combination
of such design principles and cutting-edge AI
methods promise to deliver a more rational
design of degraders.
From a proteomics perspective, the main challenge
is throughput, including MS and sample
preparation. The proteomics department at
Plexium has heavily invested in sample prep
automation, increased throughput of our
LC-MS runs and implemented a robust and
fast data analysis pipeline.
Q: How do you see the field of TPD
evolving in the next few years? What
role do you think MS will play in shaping
the future of this industry?
AC: I believe that recent developments in
proteomics sample preparation technologies
and the combination with other fields such as
biophysics are revolutionizing the TPD toolbox.
Furthermore, advances in LC-MS technology
have significantly improved the throughput,
sensitivity, precision and robustness of proteomics
workflows. The MS-based TPD toolbox
has evolved from global proteome profiling
to more functional approaches designed to
elucidate the mechanism of action of TPD.
An arsenal of approaches has been put forward
to elucidate compound-driven proteomic
changes. In recent years, we have seen a
boom of chemoproteomics and activity-based
probe profiling (ABPP) approaches, heavily
inspired by the work coming from Ben
Cravatt and Dan Nomura’s groups. I think we
will continue to see more of these methods.
For example, the streamline cysteine activity-
based protein profiling (SLC-ABPP) method
implemented by the Cravatt and Gygi groups
enables proteome-wide screening of large
fragment-based libraries in a high-throughput
fashion.
Although very powerful, chemoproteomics
and ABPP typically require the functionalization
of small molecules which can be challenging
and sometimes unwanted. The combination
of MS with other biochemical or biophysical
procedures has emerged as a powerful technology
to investigate the impact of compound
binding on protein structure. Methods such as
CETSA-MS, DARTS, LIP-MS, SPROX, HDX
and FPOP have been used in the MS-based
proteomics field for years to study ligand-protein
engagement, and we are now slowly
seeing its application in the TPD field. I hope to
see more of this to help to elucidate the mechanisms
of action of protein degradation.
Characterization of protein structure and
protein-protein interactions is essential for
understanding TPD. In this niche, I believe that
affinity purification followed by MS (APMS) will
continue to play an important role in untangling
the mechanism of action of degradation. In
particular, we are seeing an increasing application
of proximity-dependent labelling such as
BioID and APEX in TPD.
Finally, crosslink (XL)-MS complements the
arsenal of available MS-based techniques for
studying interaction, structure and dynamics of
proteins involved in degradation. XL-MS data
can help to elucidate 3D structures of small
molecule-inducible proteins and map protein
interfaces. I strongly believe that integrating
XL-MS with other techniques of structural
biology, such as cryo-electron microscopy, will
help us to address important questions in the
TPD field.
Targeted Protein Degraders 17
Ask the Experts
Summary:
In recent years, scientific research has capitalized
upon naturally occurring ubiquitin-proteasome
(UPS) and autophagy-lysosome
pathways to develop novel protein activity
inhibitors. These inhibitors, also known as
“degraders”, selectively target proteins for
destruction and hence perturb molecular
activity. Targeted protein degradation (TPD)
methods have a number of benefits over
genetic knockdown and chemical inhibition
approaches, as summarized in Figure 1.
TPD as a Powerful Research Tool
in Basic Biology and Drug Target
Discovery
Tao Wu, Hojong Yoon, Yuan Xiong, Sarah E. Dixon-Clarke, Radosław P. Nowak, Eric S. Fisch
Nat. Struct. Mol. Biol. 2020, 27, 605–614
DOI: 10.1038/s41594-020-0438-0
Figure 1
Key benefits of TPD
approaches to controlled
protein perturbation when
compared to genetic knockdown
(CRISPR) or chemical
inhibition methods.
Most current TPD methods work via the
UPS. Hence, validation techniques for the
characterization and quality control of UPS
pathways are essential in the assessment
of selectivity and degradation efficiency of
potential TPD therapeutics. By interrogating
the UPS pathway with known inhibitors (see
Figure 2) the effects of TPD modalities can be
explored using a variety of molecular biology
techniques (see Table 1).
Great temporal and
spatial control
Readily
reversible
Lower likelihood of o-target
eects
Lower chance
of molecular
compensation
Faster molecular action
Targeted Protein Degraders 18
Application Examples
Figure 2
Validation methods for
targeted protein degradation
at different stages of the
UPS pathway.
Table 1: Validation methods for TPD.
Ternary complex
formation
E1/E2/E3 cascade
Ubiquitination
MLN7243, E1 inhibitor
MLN4924, Neddylation
inhibitor
Protein
unfolding
Proteasomal
degradation
Proteasome
Bortezomib
MG-132
p97 inhibitor
E3 ligase binder p97
POI PROTAC E3 ligase Target binder
E3 ligase binder?
Target binder?
In vitro dimerization?
Cellular dimerization?
Cellular engagement?
In vitro ubiquitination?
Cellular ubiquitination?
Cellular degradation?
Recycle Degradation selectivity?
Understanding UPS pathways makes it possible to
engineer TPD systems that control protein turnover and
facilitate the study of proteins of interest (POI) in human
health and disease. Hence, by focusing on UPS pathway
and small-molecule degrader research, it is possible
to gain insights into the mechanisms driving cellular
processes and potential novel therapeutics.
Targeted Protein Degraders 19
Application Examples
Steps Purpose Example assays and chemical tools
Dimerization—in vitro Ternary complex formation in vitro TR-FRET4043, SPR/BLITS
Dimerization—cellular Ternary complex formation in cells NanoBit, NanoBret
Cellular engagement Cellular permeability and cellular engagement of
the E3 ligase
Degradation-based engagement assay
NanoBret-based fluoro-ligand displacement
Ubiquitination—in vitro Verify ubiquitination In vitro ubiquitination assay
Ubiquitination—cellular Ubiquitination quantification and type
Identify ubiquitination sites
Western blot, NanoBret, TUBES
Proteomics
Cellular degradation—targeted approach Verify ubiquitination in cells Western blot, GFP-fusion, mCherry reporter lines
Endogenous CRISPR fusions (HiBit Tag, split GFP)
Cellular degradation—selectivity profiling by
proteomics
Verify degradation selectivity in cells Proteomics approaches, library-based screens
UPS inhibitors Inhibitors of the cullin-RING family of ligases MLN494-a specific inhibitor of the NAE1/UBA3
Nedd8-activating enzyme
CSN5i-3-inhibitor of COP9 signalosome
p97 inhibitor CB-5083
Ubiquitin E1 (UBA1) inhibitor MLN7243
Proteasome inhibitors Bortezomib, carfilzomib and MG132
Tag-targeted protein degrader (tTPD) systems
direct degrader compounds to protein tags
using targeted heterobifunctional molecules
that allow rapid, reversible degradation of
tagged substrate proteins of interest (POIs).
tTPD systems targeting FKBP12F36V (dTAGs)
or HaloTag7 (HaloPROTACs) have shown
promise as preclinical validation systems, but
both have limitations and side-by-side comparisons
have not been performed.
Development of NanoLuc-Targeting
Protein Degraders and a Universal
Reporter System To Benchmark
Tag-Targeted Degradation
platforms
Christoph Grohmann, Charlene M. Magtoto, Joel R. Walker, Ngee Kiat Chua, Anna Gabrielyan,
Mary Hall, Simon A. Cobbold, Stephen Mieruszynski, Martin Brzozowski, Daniel S. Simpson,
Hao Dong, Bridget Dorizzi, Annette V. Jacobsen, Emma Morrish, Natasha Silke, James M.
Murphy, Joan K. Heath, Andrea Testa, Chiara Maniaci, Alessio Ciulli, Guillaume Lessene, John
Silke & Rebecca Feltham
Nat commun. 2022, 13 (2073)
DOI: 10.1038/s41467-022-29670-1
FKBP12F36V
Tagged POI
POI
NanoLuc
HaloTag
Tag-targeting
Tag degrader
Tag-POI CRL
ternary complex
CRL E3
complex
POI
degraded
Ub
+ =
Tag
Figure 1. Development of NanoTACs: a NanoLuc-targeting degrader system. a) Schematic depicting the tTPD systems. FKBP12F36V, NanoLuc or Halo
epitope tags are fused to POI and tag-targeting heterobifunctional degrader compounds are employed to hijack cullin-RING ligase (CRL) complexes to trigger
proteasomal degradation of the tagged POI. b) Comparison of tools and properties of each tag for tag-targeted protein degradation. CRBN, cerebelon; VHL, Von
Hippel-Lindau; IAP, inhibitors of apoptosis protein; NT, not tested.
a)
b)
Targeted Protein Degraders 20
Application Examples
FKBP12F36V HaloTag and NanoLuc Tag Comparison
Tag MW (kDA) CRBN
degrader
VHL degrader IAP degrader Catalytic
degrader
Antibodies Luminescence
properties
In vivo activity
FKBP12F36V 12 Yes Yes X Yes Yes X Yes
Nanoluc 19 Yes X X Yes Yes Yes NT
HaloTag 33 X Yes Yes X Yes X Yes
NanoLuc is a bioluminescent protein tag with
multiple advantages over other tags such as
stability, catalytic properties, small size and
ease of availability. In this work, NanoLuc-targeting
PROTACs (NanoTACs) were developed
to expand the tTPD repertoire and trigger
proteasomal degradation of NanoLuc tagged
substrates. The properties of the NanoLuc
reporter system were assessed in comparison
with dTag and HaloPROTACS (Figure 1a,
1b).
A stable, universal tTPD reporter protein containing
all three protein tags (Halo, FKBP12F36V
and NanoLuc) was synthesized to enable
comparative studies (Figure 2). The addition
of a Firefly luciferase enabled direct comparison
of all tTPD systems by luminescence.
Using the H-FF-N-F reporter system, multiple
HaloPROTACs, NanoTACs and FKBP12F-
36CV-targeting degraders were assessed for
degradation capabilities. Although the NanoLuc-
CRBN targeting NanoTac, NC4, could
trigger efficient degradation, the FKBPF36V- targeting
(dTag) system was seen to trigger the
most efficient substrate degradation.
Additionally, the ability of the tTPD system
to degrade a biologically relevant, tagged
protein was examined. Both the NanoTAC
NC4 and the FKBPF36V-targeting degrader
FV1 induced degradation of pro-necroptotic
pseudokinase MLKL, to a level sufficient to
prevent necroptotic cell death. Importantly,
the global proteomic analysis demonstrated
that NC4 triggers specific degradation of the
target substrate with no significant off-target
degradation observed when assayed against
5591 proteins
Overall, FKBPF36V tTPD systems are the
most efficient and fastest platforms for
degrading tagged substrates. However, the
addition of NanoTACs to the tTPD repertoire
adds further flexibility to tTPD studies.
Together, NanoLuc and other tTPD systems
will be a crucial part of future validation studies
on prospective therapeutic targets.
HaloTag
Firefly
NanoLuc
FKBP
F36V
Halo-Firefly-NanoLuc-FKBPF36V (H-FF-N-F)
Figure 2
Schematic depicting the
Halo-Firefly-NanoLuc-FKBPF36V
(H-FF-N-F) fusion
protein.
Targeted Protein Degraders 21
Application Examples
Online information
bruker.com/sc-xrd
Targeted Protein Degraders 24
Bruker Switzerland AG
Fallanden Switzerland
Phone +41 44 825 91 11
ms.sales.bdal@bruker.com - www.bruker.com
Bruker Scientific LLC
Billerica, MA - USA
Phone +1 (978) 663-3660
Online information
bruker.com/timstof-ultra
For Research Use Only. Not for use in clinical diagnostic procedures
Brought to you by
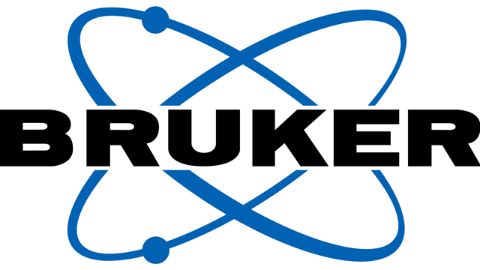
Download this eBook for FREE Now!
Information you provide will be shared with the sponsors for this content. Technology Networks or its sponsors may contact you to offer you content or products based on your interest in this topic. You may opt-out at any time.